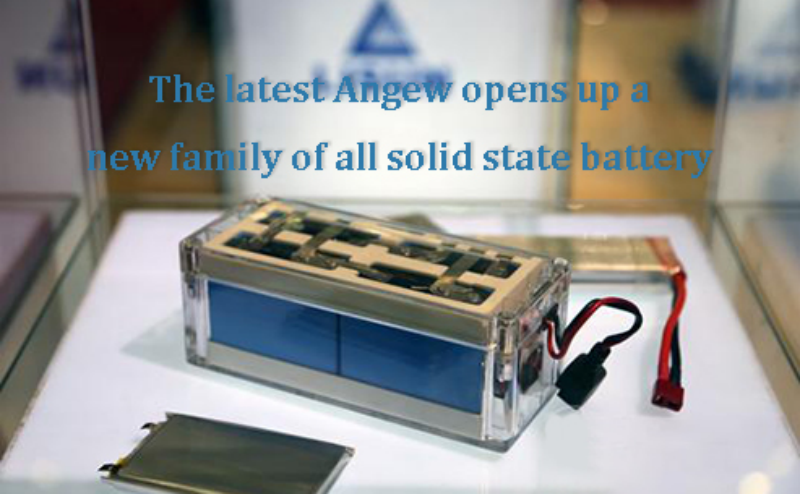
Main content:
1.Summary of the full text
Achieving fast Li-ion transport at room temperature is a major challenge for all solid state battery with large battery capacity. Metal borohydrides with neutral ligands are an emerging class of solid-state ionic conductors, and a team from Aarhus University in Denmark reports the discovery of a novel monomethylamine lithium borohydride with very fast Li+ conductance at room temperature. LiBH4∙CH3NH2 is a monoclinic space group P21/c with a two-dimensional unique layered structure.
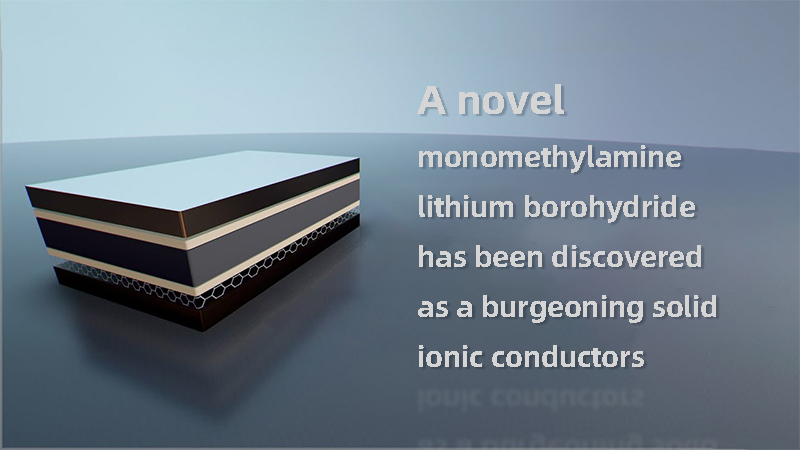
The layers are separated by hydrophobic -CH3 moieties and contain large voids, enabling fast Li-ion conduction in the interlayer with σ(Li+) = 1.24∙10-3 S/cm at room temperature. The electronic conductivity is negligible and the electrochemical stability is ~2.1 V vsLi. The team investigated the first all solid state battery using lithium borohydride with neutral ligands as the electrolyte, lithium metal as the anode, and TiS2 as the cathode. The research was published in the top journal "AngewandteChemie" in the field of chemistry with a paper titled "MethylamineLithium Borohydride as Electrolyte for All-Solid-State Batteries".
2.Research highlights
1.The effects of neutral molecules containing hydrophobic moieties are studied and the first member of a new class of borohydride compounds is reported - methylamine metal borohydrides. 2.The synthesis and crystal structure of lithium methylamine borohydride LiBH4∙CH3NH2 are reported, which exhibits extremely high Li+ conductivity at room temperature, making it a promising solid-state electrolyte.
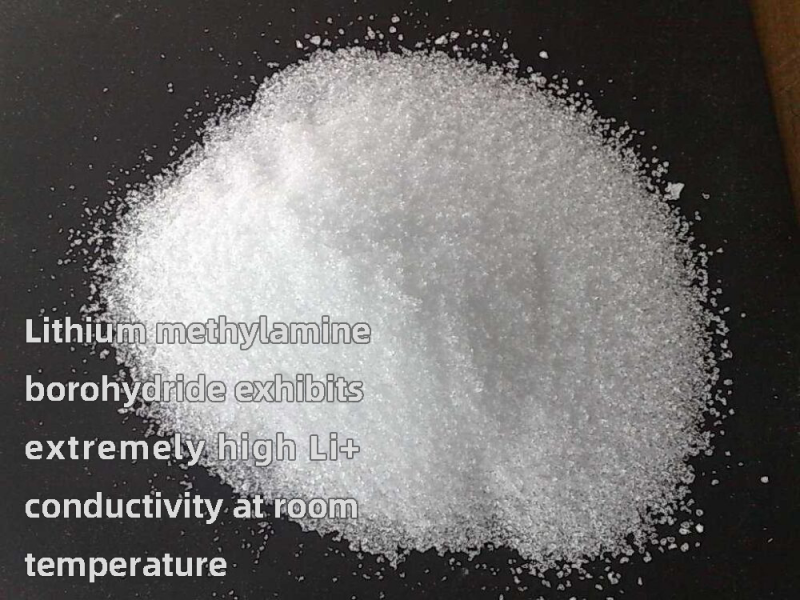
3.The compound was characterized by powder X-ray diffraction (PXD), 11B magic angle spinning nuclear magnetic resonance (11BMASNMR) spectroscopy, Fourier transform infrared spectroscopy (FTIR), and thermal analysis, and electrochemical properties were tested using electrochemical impedance spectroscopy ( EIS), cyclic voltammetry (CV), and galvanostatic cycling (GC). An all solid state battery was also assembled using a lithium metal anode and a layered TiS2 cathode.
3.Introduction to the text
LiBH4∙CH3NH2 was prepared by a gas-solid reaction between LiBH4 and CH3NH2, and then the excess CH3NH2 was removed by vacuum evacuation. FTIR revealed new absorption patterns and assigned based on related compounds in the literature. LiBH4∙CH3NH2 shows similar B-H stretching (2000-2500 cm-1) and bending (1000-1400 cm-1) modes as LiBH4. The new modes assigned to CH3NH2 are considered as N-H stretching (3300 and 3350 cm-1), C-H stretching (2750-3000 cm-1), N-H bending (1460 and 1600 cm-1) modes, and regions of 850-1350 cm-1 Contains B-H bending, C-H bending, CH3 rocking and C-N stretching modes. Weak NH2 rocking modes were observed at 680–760 cm-1 with significantly lower intensity compared to molecular CH3NH2, most likely due to the restricted movement from ligands to Li+ in the crystal structure. LiBH4∙CH3NH2 is hygroscopic, and water in contact with air reacts slowly, and all vibrational modes corresponding to BH4- and CH3NH2 disappear after 24 h.
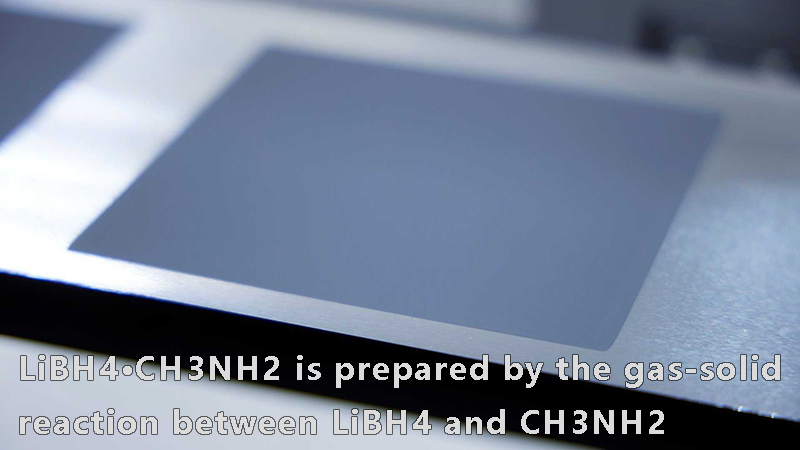
① Crystal texture of LiBH4∙CH3NH2
The SRPXD data show that all Bragg reflections corresponding to LiBH4 disappear at T=-23°C, and the observed Bragg reflections can be observed at T=-23°C with space group symmetry P21/c and unit cell parameters a=9.1972(2), b = 7.2148(1) is indexed in the monoclinic unit cell, c=7.1157(1)Å, β=96.4930(5)° and V=469.14(2)Å3, indicating the composition LiBH4∙CH3NH2 with Z=4. The structure was solved using ab initio and the final Rietveld refinement provided a convincing fit to the observed SRPXD data.
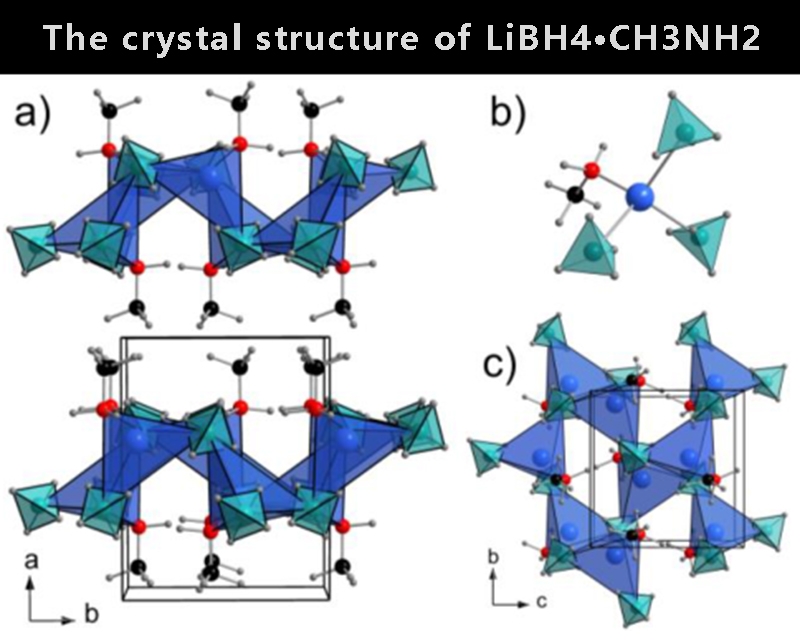
【Figure 1】The crystal structure of LiBH4∙CH3NH2. a) Layer viewed from the ab plane, b) local lithium coordination and c) layer viewed from the bc plane.Match colors: Li+ (blue), BH4- (light blue tetrahedron), N (red), C (black) and H (gray)
In the structure of LiBH4∙CH3NH2 (Fig. 1a), a unique Li site is coordinated by three BH4- and one CH3NH2 to form [Li(CH3NH2)(BH4)3] tetrahedral units (Fig. 1b). The BH4-anion coordinates to the two Lis via edge common (κ2) with a twisted coplanar (κ3), resulting in a Li coordination number of 8. [Li(CH3NH2)(BH4)3] tetrahedral units bridge the BH4-groups through edge- or corner-sharing to form 2D layers in the bc plane (Fig. 1c), which stack along the a-axis. The layers are interconnected by hydrophobic interactions between -CH3 groups. The Li-N distance is 2.05 Å and the Li-B distance is in the range of 2.49–2.67 Å, similar to the observations for LiBH4∙NH3.However, the structural morphology is different from that of LiBH4∙NH3, which forms a one-dimensional chain.
Analysis of voids in the crystal structure revealed large cavities along the c-axis, forming a preferred one-dimensional pathway large enough to accommodate interstitial-migrating Li+ ions. These cavities are connected along the b-axis unit cell edge to form a two-dimensional conduction pathway. A preferred one-dimensional conduction path is shown in Figure 2, where the conduction path of interstitial Li+ ions is a zigzag pattern following the positions of the BH4- groups. The BH4- groups may be reoriented to stabilize the coordination of framework and transport interstitial Li+, while CH3NH2 may be exchanged between two Li+ ions, similar to the reported conduction of similar compounds LiBH4∙½NH3 and Mg(BH4)2∙NH3 mechanism.
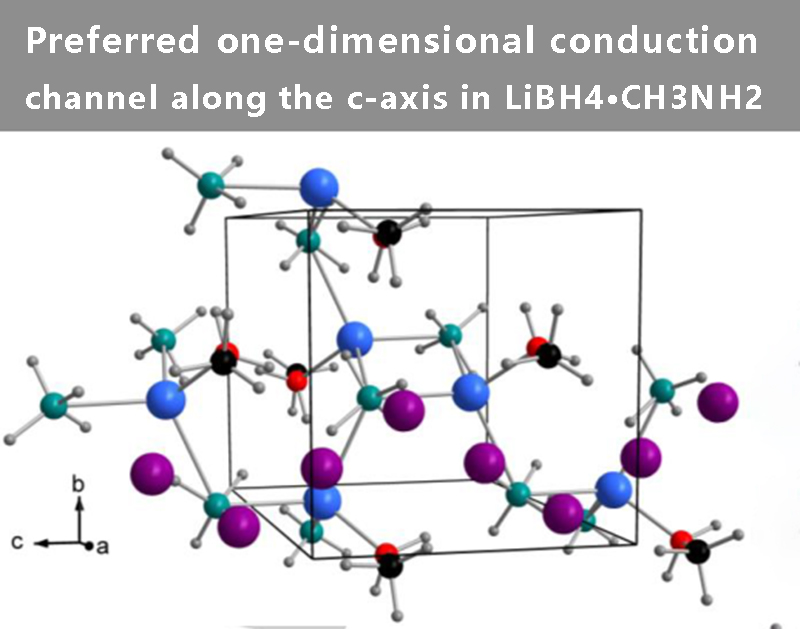
【Figure 2】Preferred one-dimensional conduction channel along the c-axis in LiBH4∙CH3NH2. Color matching: Interstitial Li+ (purple), Skeletal Li+ (blue), BH4- (light blue tetrahedron), N (red), C (black), and H (grey)
The 11B (I=3/2) MASNMR spectra of LiBH4∙CH3NH2 at its center (m=½↔m=-½) and satellite (M =±½↔ M =±3/2) transitions (Figure 3A) are derived from the circulation of the unique boron site at the center and spin side bands (SSBS) in LiBH4∙CH3NH2. Furthermore, the isotropic chemical shift of 11B is δiso = -40.5 ppm, revealing the presence of BH4-units, which are clearly different from the corresponding borohydride units in o-LiBH4. δiso=-41.0ppm, the spectrum obtained under the same experimental conditions is consistent with the previous 11BNMR study. The manifold of ssbs can be modeled by considering the mutual existence of quadrupole coupling interactions and chemical shift anisotropy (CSA) using methods described in ref.
The least squares fit of the simulated to experimental SSB intensities gives the optimized simulation shown in Fig. 3b, corresponding to the quadrupole coupling parameters, CQ=98±8kHz and ηQ=0.42±0.03, δiso=-40.5±0.1 ppm, δσ=17±4ppm and ησ=0.56±0.30, and the relative directions of the two tensor interactions defined by Euler angles at 12.0°C ψ=180°, χ=37°±25° and ξ=0°. These parameters are very similar to the corresponding values reported for o-LiBH4 at 25°C (δiso = 41.0 ppm, CQ = 99 kHz, ηQ = 0.91, δσ = 30 ppm, ησ = 0.91), indicating that BH4- The local geometry or dynamics of the elements are similar. The 13C{1H} MASNMR spectrum of LiBH4∙CH3NH2 shows a single resonance at 28.5 ppm, which is in good agreement with the 13C chemical shift of liquid CH3NH2.
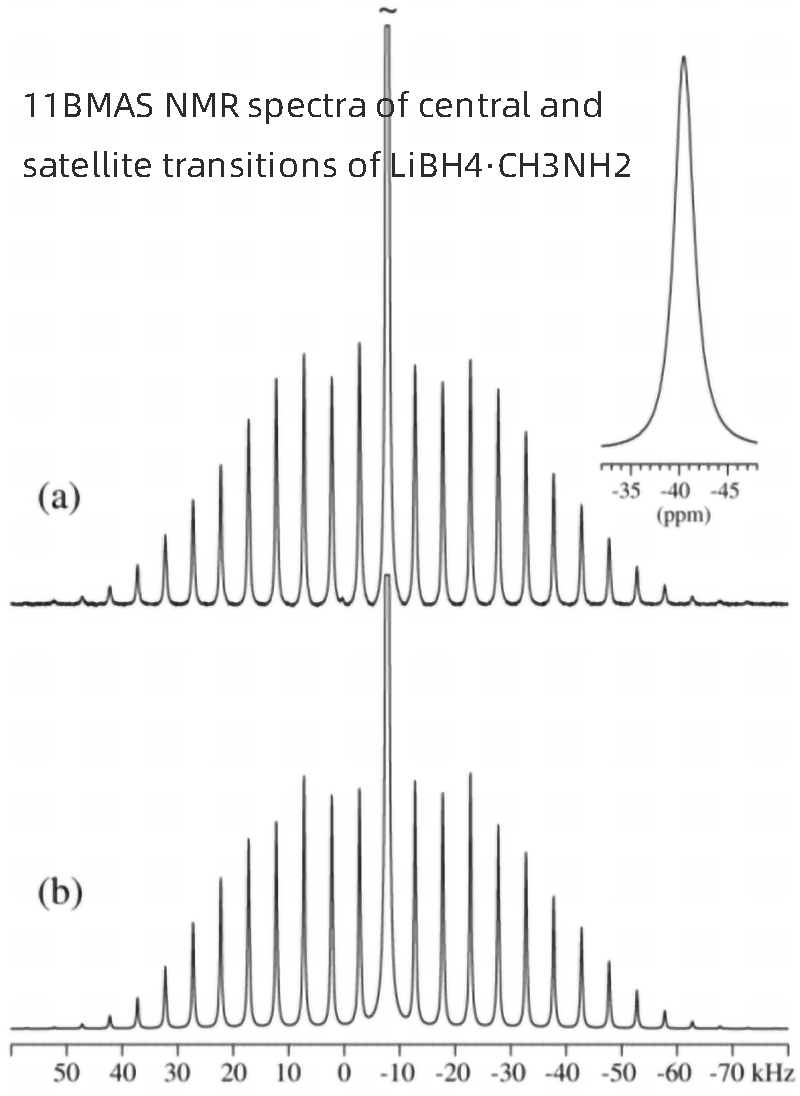
【Figure 3】a) 11BMAS NMR spectra of the central and satellite transitions of LiBH4∙CH3NH2, obtained at 14.09 T using a rotational speed of υR = 5.0 kHz and the sample at 12.0 °C. The inset illustrates the center band resonance. b) An optimized simulation of the spectra in (a), corresponding to the 11B interaction parameters given in the text
② Thermal analysis
Figures 4a and 4b show the in situ SRPXD and TG-DSC-MS data of LiBH4∙CH3NH2 during heating.All Bragg reflections correspond to LiBH4∙CH3NH2 and disappear at 45 to 50 °C, after which the sample becomes amorphous. No change was observed during cooling. The endotherm at 44°C and visual inspection indicated melting of the sample and marked the beginning of the gradual release of CH3NH2 and a small amount of H2.
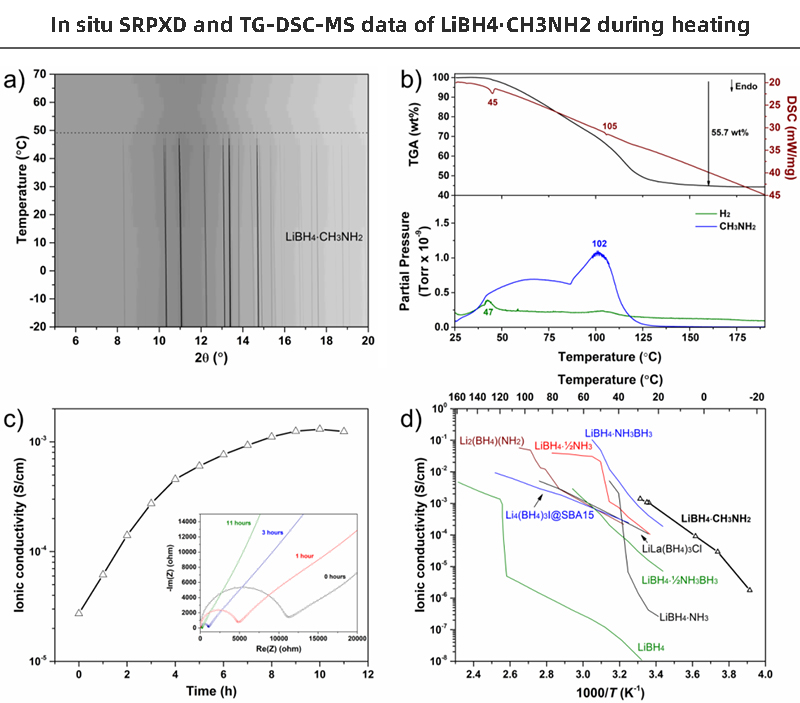
【Figure 4】a) In situ SRPXD data (λ=0.824958Å, 5°C/min) .b) TG-DSC-MS data of LiBH4∙CH3NH2 (0.5°c/min). c) Time dependence of Li+ conductivity at T = 24°C and selected Nyquist plots (inset). d) Temperature dependence of Li+ conductivity compared with other selected LiBH4 derivatives
The second endotherm at 105°C coincides with the increased release of CH3NH2 and may be related to the orthorhombic to hexagonal polymorphic transformation of LiBH4. A total mass loss of 55.7 wt% was observed upon heating to 120 °C, which is in good agreement with the expected mass of one CH3NH2 molecule per formula unit (58.8 wt%, calculated).
③ Electrochemical characterization
Li+ conductivity was tested using EIS measurements of symmetric cells with Mo barrier electrodes Mo|LiBH4∙CH3NH2|Mo (Fig. 4c,d). At room temperature, the cell resistance rapidly drops by almost two orders of magnitude over time. This indicates a decrease in interfacial resistance within the particles or in the grain boundaries towards the Mo electrode. To ensure that the effect was not a reaction with air, tests were also performed in a glove box, which also showed a decrease in resistance over time. The cell resistance stabilized after 10 hours, after which the temperature-dependent Li+ conductivity was tested in the low temperature range from T = -18 to 29°C to avoid any decomposition of the samples. The Li+ conductivity of LiBH4∙CH3NH2 is the highest among all known LiBH4 derivatives, and its conductivity is more than five orders of magnitude higher than that of pristine LiBH4 at room temperature (Fig. 4d).
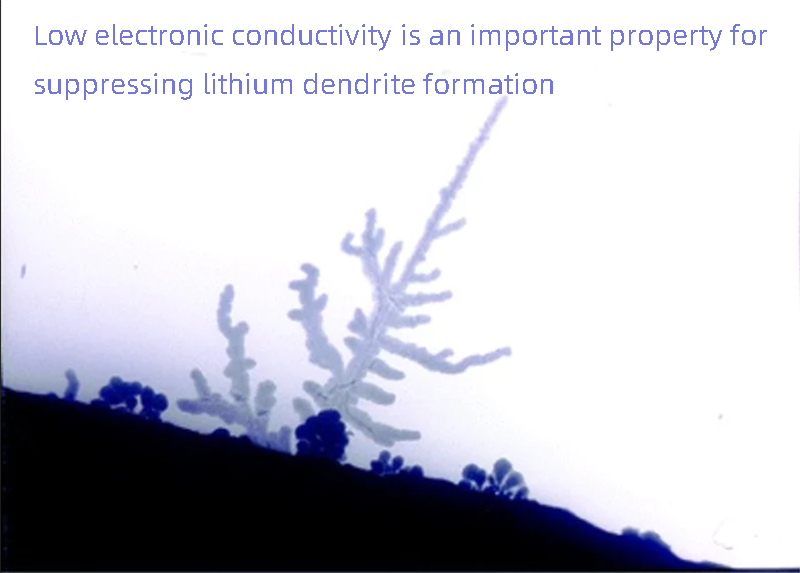
More importantly, the Li+ conductivity is high enough for the battery to operate under ambient conditions, σ(Li+) > 10-3S/cm. The activation energy for Li+ conduction is similar to several other LiBH4 derivatives, with Ea = 0.83 eV over the temperature range T = -6 to 29 °C, while the conductivity at T = -18 °C appears to be slightly lower than the linear Arrhenius expectations of behavior. Use DC voltage polarization of a symmetric cell with blocked electrodes to verify that high conductivity is not electronic. Peak current (iT) to 0.5V polarization leads (Fig. 4c), resulting in an increase in conductivity from σ(Li+) = 2.73∙10-5 S/cm to 1.24∙10-3 S/cm for 10 hours, an increase of 4.18∙10 -5 μA cm-1 current response and 1.36∙10-8 μA cm-1 steady-state current.According to the relationship of tion=iion/iT=(iT-ie)/iT, the ion migration number (tion) is estimated to be 0.9983. Low electronic conductivity is an important property for suppressing dendrite formation, and similarly low values were found for pure LiBH4.
The electrochemical stability of LiBH4∙CH3NH2 was investigated with CV in a two-electrode setup with Mo as the working electrode and Li as the counter and reference electrodes (Fig. 5a). At low potentials, Li deposition/stripping on Mo electrodes was observed below and above 0V, respectively, and the current increased with the number of cycles. This indicates an improved contact or interface between the electrolyte and Li metal during cycling. The anode current starts to flow at 2.1 V, corresponding to the oxidation of the BH4-anion, as previously predicted from DFT calculations and consistent with the situation for other LiBH4-based electrolytes. Furthermore, a small oxidation current flows at 1.2 V, which may be responsible for the current fluctuations (spikes) observed at higher cycle numbers, such as the 10th cycle.
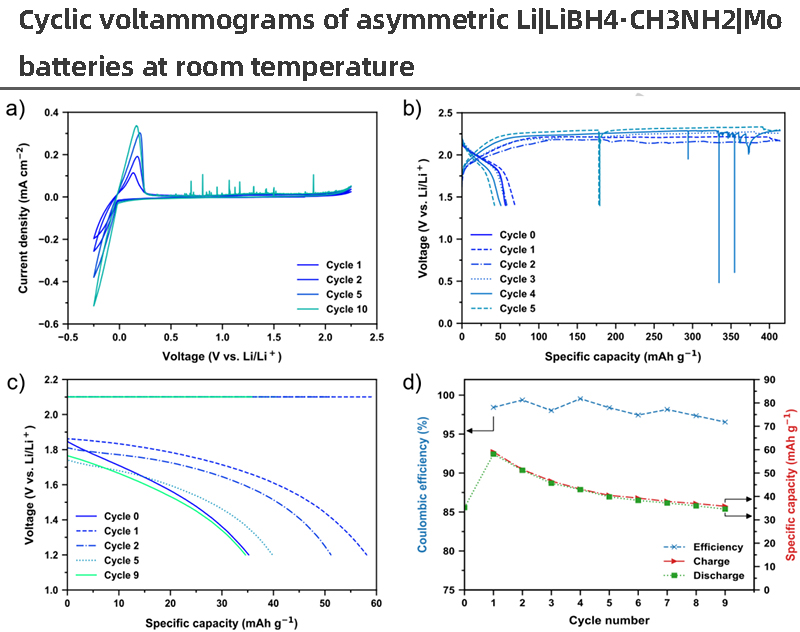
【Figure 5】 a) Cyclic voltammograms of an asymmetric Li|LiBH4∙CH3NH2|Mo battery at room temperature with a scan rate of 1.0mV/s and a potential range of -0.25V to 2.25V. b) Galvanostatically cycled all solid state battery with Li|LiBH4∙CH3NH2|TiS2 (cathode composition: TiS2:LiBH4∙CH3NH2=0.65:0.35wt%) at C/20 (46 μA/cm2) with a cut-off voltage of 1.4 V (and 2.4V), the cut-off time is 30 hours.c) An all solid state battery with Li|LiBH4∙CH3NH2|TiS2 (cathode composition: TiS2:LiBH4∙CH3NH2=0.78:0.22wt%) was charged at a constant current/voltage of 2.1V with a limited current of 10μA and C/20 (110μA· cm2) constant current discharge, the cut-off voltage is 1.2V. d) Charge (red)/discharge (green) capacity and Coulombic efficiency as a function of cycling for the cells shown in c). All electrochemical characterizations were performed at T = 30 °C
An all solid state battery with LiBH4∙CH3NH2 as the solid electrolyte and TiS2 as the cathode was assembled and tested using CV and GC (Fig. 5b). TiS2 were chosen as the cathode materials because of their potential (~2 V) in the electrochemical stability range of LiBH4∙CH3NH2, and it is a well-studied lithium battery cathode. The lithiation and delithiation in TiS2 were observed from the voltammogram, but the current from 2.1V was much higher than the irreversible anode current for the delithiation of TiS2. A similar situation exists in the charge/discharge curves when the C rate is C/20 during galvanostatic cycling of the same cell, that is, oxidation occurs at 2.2 V until the charge step is cut off after 30 h. The maximum discharge capacity (2nd discharge) resulted in a capacity of 69 mAh/g, which is ~30% of the theoretical capacity assuming one Li per TiS2.
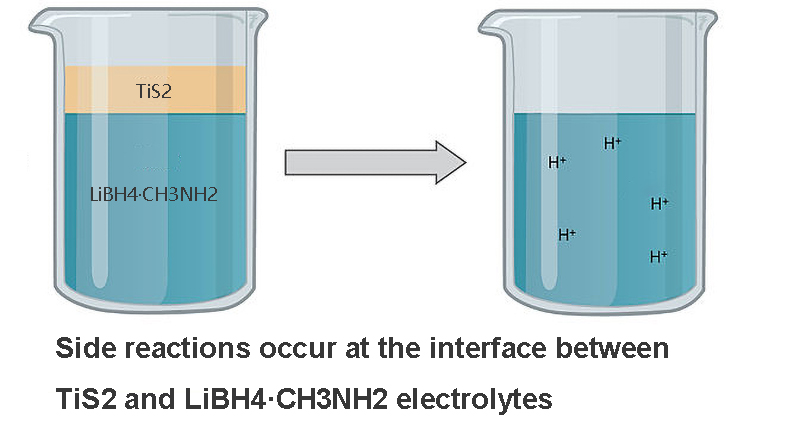
These results indicate that side reactions occur at the interface between TiS and LiBH4∙CH3NH2 electrolytes due to the limited oxidative stability of the BH4-anion (<2.1 V). As a comparison, it has been reported that the cycling in Li|LiBH4|TiS2 solid-state batteries is more stable, possibly due to the formation of a stable Li2B12H12-containing solid electrolyte interface. This was observed as a low initial discharge capacity due to self-discharge upon interfacial layer formation, while higher discharge capacity and more stable cycling were subsequently observed. Therefore, this suggests that the LiBH4∙CH3NH2 electrolyte forms a different and unfavorable interface, which cannot protect the electrolyte from further oxidation.
To avoid this side reaction, an additional cell was investigated with a constant voltage charge of 2.1 V and a constant current discharge of 10 μA and C/20 (Fig. 5c). The cell has a larger separator, which results in a higher overpotential and higher TiS2 loading. However, the performance of this cell resulted in higher Coulombic efficiency (~98%) but similar capacity (Fig. 5d), suggesting that side reactions can be reduced by adjusting the cycling program. Therefore, in high-voltage battery applications, the use of LiBH4∙CH3NH2 as the electrolyte depends on forming a more favorable interface to reduce the voltage gradient in the electrolyte.
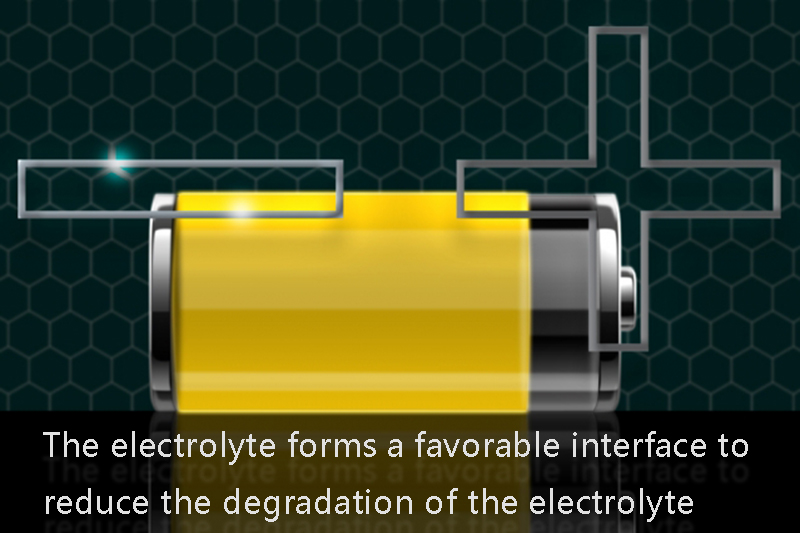
Nano-confined LiBH4 and its derivatives have previously shown improved battery stability due to the formation of a favorable interface. Such an interface requires very low electronic conductivity to minimize electrolyte degradation. Alternatively, the electrolyte may be used in combination with a positive electrolyte, ie an electrolyte that is stable to the positive material, or by coating the positive material. These methods have been investigated for thiophosphate and lithium magnesium sulfate electrolytes, which also have relatively low oxidative stability.
4.Summary and outlook
In conclusion, LiBH4∙CH3NH2, the new compound for all solid state battery is reported. It exhibits the highest room-temperature Li-ion conductivity observed in LiBH4-based materials, with σ(Li+)=1.24∙10-3S/cm at 24°C. The neutral ligand CH3NH2 was introduced to create a two-dimensional monoclinic structure.The structure consists of layers in the bc plane, consisting of [Li(CH3NH2)(BH4)3] tetrahedral units linked by bridging borohydride groups. The structure contains voids in the interlayer, forming a preferred one-dimensional lithium ion conducting channel along the c-axis, which is connected to the two-dimensional conducting network through the voids along the b-axis. Electrochemical characterization shows that, similar to other LiBH4-based materials, the oxidative stability on the Mo electrode is about 2.1 V (vs. Li) and reversible deposition/stripping.
The first LiBH4 proof-of-concept cell with neutral ligands was demonstrated using a TiS2 cathode, reaching a maximum discharge capacity of 69mAh/g at 30°C. The oxidative degradation of the electrolyte is suppressed (>2.1V) by constant current/constant voltage charging at 2.1V, and the Coulombic efficiency is about 98%. This work demonstrates a new fast lithium-ion conductor electrolyte that allows all solid state battery to operate under ambient conditions. Stabilization of high electrical conductivity to even lower temperatures can be achieved by mixing with previously reported nanoparticles, such as with MgO and Al2O3, and further screening of compatible cathode or catholyte materials may be beneficial for the development of new high-energy all solid-state lithium-ion battery.