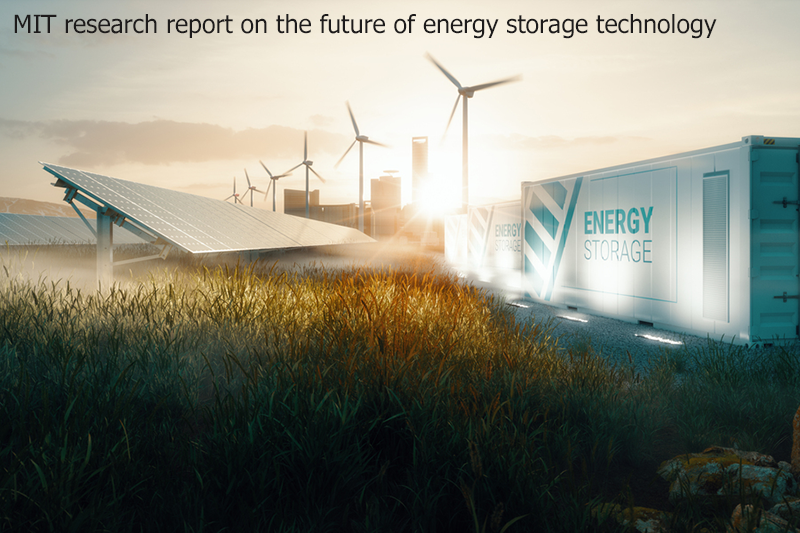
Main content:
- Preface
- Summary
- Basic knowledge of multiple energy storage technologies
- Energy storage technology used in power system
- Electrochemical energy storage
- Thermal energy storage
- Chemical energy storage
- Mechanical energy storage
- Efficient renewable energy power systems with energy storage
- Modeling results for developed countries: three regions in the United States
- Modeling results for emerging market and developing economies: India
- Other research topics
1. Preface
The future of energy storage technology is the ninth research project in the MIT Energy Program's Future of Research series, which aims to shed light on a range of complex and important issues involving energy and the environment. The first few research projects have focused on the important role of energy technologies such as nuclear power, solar power, natural gas, geothermal and coal (carbon capture and sequestration of carbon dioxide emissions) and the U.S. electrical grid system.At the heart of these research projects is understanding the important role these specific technologies can play in decarbonizing the global energy system and meeting future energy demands.
As a high-performance energy storage battery technology, lithium ion solar battery makes full use of solar energy and improve the photoelectric conversion efficiency. Energy storage will play an important role in achieving both goals by complementing variable renewable energy sources such as solar and wind power, resources that are central to decarbonizing the power sector. The research project will provide reference and assistance to governments, industry and academia as these sectors and institutions develop emerging energy storage industries and consider changes to power sector planning, oversight and regulation that will significantly increase interest in variable renewable energy deployment and reliance on energy storage systems.
The report is the culmination of more than three years of research into energy storage technologies - including opportunities to develop low-cost long-duration energy storage, and through modeling studies to assess future deep decarbonization of the U.S. region and emerging market and developing economy countries. Types and roles of energy storage technologies as renewable energy generation continues to grow in the grid and the impact of energy storage systems on power system planning and regulation. This research project was initiated and conducted at the research institute MIT Energy Initiative (MITEI) and was guided by an MIT researcher-based advisory board whose members participated in several workshops.
2. Summary
The report of this interdisciplinary study released by MIT examines the important role energy storage systems will play in the future as the decarbonization of the power system becomes central to combating climate change, noting the deep decarbonization of the power generation system and multiple Industry electrification is necessary to limit climate change and its damage. The report notes that wind power and solar power are significantly cheaper and are being deployed on a large scale around the world, and are likely to account for a large share of total future power generation.
Unlike traditional power generation facilities, the power output of these variable renewable energy sources (VREs) is dependent on weather conditions, which can sometimes change rapidly, making it difficult for renewable generation to be dispatched to meet changes in power demand. Energy storage technology, which is the focus of this research report, can play a key role in balancing electricity supply and demand, and can provide other services needed to keep decarbonized power systems reliable and cost-effective.
As the researchers discuss in this report, energy storage systems comprise a range of technologies that differ in material requirements and value in low-carbon power systems. With the large-scale deployment of energy storage systems on the grid, policies must be adjusted to avoid excessive and unfair cost burdens on consumers, electrification or economy-wide decarbonization needs to be encouraged, and strong economic growth is achieved, especially in Emerging market and developing economies. Social justice and equity must be included in system design.
The time span of this research project has been extended to 2050, consistent with previous studies in its Energy Plan Futures series, but also of interest in energy technologies that could be deployed on a large scale in the closer 2030 time frame. Energy storage helps the power system achieve cost-effective decarbonization goals, and will rely more on wind and solar power without sacrificing power system reliability.
Assuming that favorable trends in variable renewable energy (VRE) cost reductions continue, modelling analyses conducted by this research project identify cost-effective pathways for decarbonizing the power system—for example, relative to carbon emissions in the United States in 2005 reduce emissions by 97% to 99% while maintaining grid operational reliability.
Efficient decarbonization of the grid requires significant investment in multiple energy storage technologies, as well as in transmission, clean generation and demand flexibility. If “negative emissions” technologies (that is, technologies that remove carbon dioxide from the atmosphere) are employed, then small gas-fired power generation facilities will also be part of a cost-effective net-zero power system.
3. Basic knowledge of multiple energy storage technologies
There are currently four basic types of energy storage technologies (electrochemical, chemical, thermal and mechanical) at different levels of technological development. Energy storage systems can play an important role both when variable renewable energy (VRE) generation is plentiful and the wholesale supply price is relatively low, and when there is insufficient generation and the wholesale price is relatively high. This flexibility of energy storage systems provides a range of benefits to the power system.
The size of an energy storage system can be characterized by its installed capacity (maximum instantaneous power), measured in megawatts (MW). Its energy storage capacity is measured in megawatt-hours (MWh), and round-trip efficiency (RTE) is used to measure the efficiency of charging and discharging. The ratio of the energy storage capacity to the installed capacity of an energy storage system is the duration, measured in hours—this is the length of time that the energy storage system provides maximum power from a full charge.
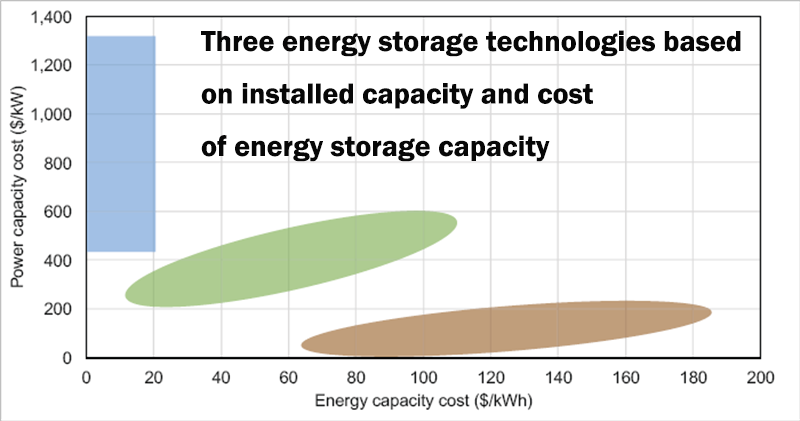
Figure 1 Three energy storage technologies based on installed capacity and cost of energy storage capacity
Most currently deployed battery storage has a duration of 4 hours or less, and most existing pumped storage (PSH) facilities have a duration of 8 to 12 hours or more. Energy storage technologies also vary in energy density (energy density is the maximum amount of energy that can be stored per unit volume). Battery technology with high energy density is particularly suitable for electric vehicles (EV) and mobile electronics. Still, battery technologies with lower energy density can be used for storage in power system applications.
In these applications, however, efficient use of space is often less important. Energy storage technologies also differ in other attributes, including the degree of economies of scale (geographical footprint and modularity) of a given energy storage system and the degree to which its performance degrades with use. The technologies considered in this report fall into three broad categories based on their power and energy capacity costs (see Figure 1). Generally speaking, energy storage technologies with lower cost of energy storage capacity and higher cost of installed capacity (blue area in the diagram) are best suited for long-term energy storage (duration of up to several days).
These energy storage technologies are charged and discharged less frequently, such as thermal energy storage, chemical energy storage, metal-air batteries and pumped hydro storage facilities. Energy storage technologies in the brown area, which include lithium-ion battery energy storage systems, are better suited for shorter duration energy storage applications (several hours in duration) and more frequent charge and discharge. Medium-capacity energy storage technologies, including flow batteries, are in the green zone.
4. Energy storage technology used in power system
This research investigates and studies energy storage technology from four aspects: electrochemical, thermal energy storage, chemical energy storage and mechanical energy storage. But not all options in these categories have been catalogued, let alone evaluated. Instead, the researchers focused on examples of energy storage technologies within each category and attempted to highlight issues with the application of these energy storage technologies.
Some of the energy storage technologies considered by the research team are proven for commercial deployment, such as lithium-ion battery energy storage systems, pumped hydro power generation facilities and some thermal energy storage systems. Other energy storage technologies require further research, development and demonstration, and may not be commercially available or applied on a large scale until the 2030s or 2040s. Table 1 summarizes the assessment of the near-term (to 2030) availability of various energy storage technologies and energy storage support technologies and practices. The energy storage technologies considered in this research report are all likely to be in commercial operation by 2050.
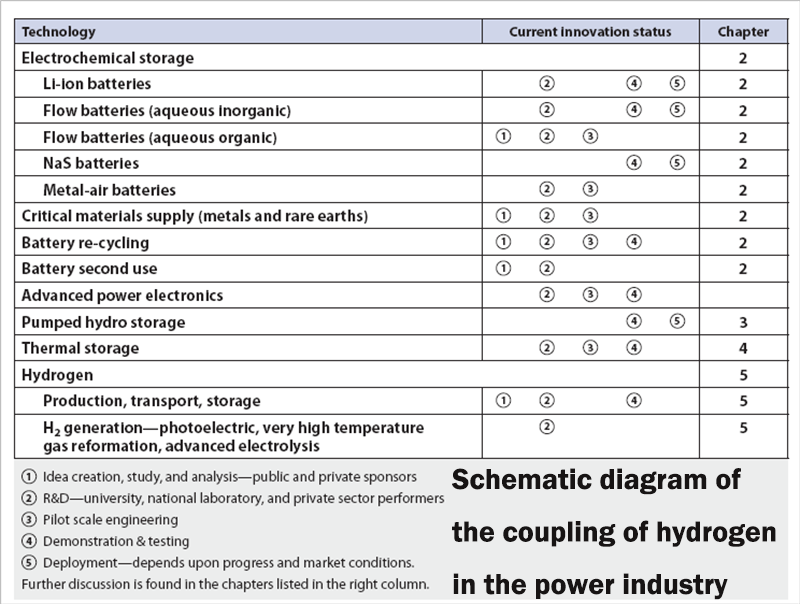
Table 1 Summary of survey results on the current state of innovation in selected energy storage technologies
Successful innovation in energy technology and other manufacturing-related technologies typically goes through five stages: ideation, R&D, pilot testing, demonstration and testing, and deployment. Table 1 presents the current stage of various energy storage technologies. Private companies have provided significant venture capital in energy storage technologies, particularly lithium-ion batteries for cars. As discussed in this research project, the development of electric vehicle batteries has significantly improved the adoption of battery energy storage systems in power systems.
So far, long-duration energy storage technology has not been similarly helped by other market drivers. Long-duration energy storage systems (duration greater than 12 hours) are less valuable when variable renewable energy (VRE) penetration is low, but as decarbonization requirements become more stringent and demand for VRE (as reliance on VRE) for power generation increases, long-duration energy storage technologies clearly become more valuable. This is especially true if grid operators are phasing out natural gas-fueled generation facilities (with or without carbon capture and storage).
The value that long-duration energy storage can provide in a highly decarbonized power system suggests that governments should increase their support for various long-duration energy storage systems, depending on the stage of innovation achieved by different technologies. The current policy focus on relatively short-term decarbonization goals has prompted both public and private attention to downstream technology demonstration and deployment involving relatively mature technologies. The U.S. Department of Energy (DOE) can play a useful role in this area, but its involvement should reflect two important lessons learned from past demonstrations and deployments.
First, the U.S. government should allow more industry-joint technology demonstration projects, free from the Federal Acquisition Regulations and other commercial terms that limit technology development and demonstration. Public investment in technology demonstration and early deployment activities aimed at disseminating knowledge is inconsistent with policies such as requiring cost-sharing in exchange for intellectual property. Second, efforts to accelerate the deployment of any commercial technology should rely on incentives and mechanisms that reward success without interfering with project management.
The U.S. government has proposed tax credits for a range of energy storage technologies, in addition to tax credits for transmission and a variety of clean generation technologies, including wind and solar. Generation technologies can link performance-based payments (such as production tax credits) directly to generation metrics, and performance-based support for non-generation energy technologies such as energy storage systems must be based on pre-set development and operational testing measures.
5. Electrochemical energy storage
Electrochemical energy storage systems include existing batteries discussed in this research project as well as new batteries, and generally have higher energy densities than mechanical and thermal energy storage systems, but lower energy densities than chemical energy storage systems. The charge-discharge round-trip efficiency of battery energy storage systems ranges from 95% for lithium-ion battery energy storage systems to 40% for metal-air chemical battery energy storage systems.
The compact footprint and independence of hydrological and geological resources make batteries a versatile and highly scalable energy storage technology that can be sized for a variety of energy storage applications from power plants to residential buildings. The study therefore draws several key conclusions. Lithium-ion battery as a long lasting battery with high energy density, high power density and high round-trip efficiency, which facilitate their almost ubiquitous use in electric vehicles and in short-term energy storage (usually lasting 4 hours or less) application scenarios widely used in.
The dominance of lithium-ion batteries in the fast-growing electric vehicle market has attracted substantial investment from the private sector and supported the rapid expansion of U.S. battery manufacturing capacity. The current price and availability of key materials used in battery fabrication lead to higher costs for lithium-ion batteries that may limit future deployment, motivating developers to move to more element-abundant chemistries. Other advancements that are being vigorously pursued in lithium-ion batteries will also support cost and performance improvements.
Given these trends, lithium-ion batteries will continue to be the leading technology for electric vehicles and short-term energy storage systems. But in terms of energy storage capacity, its cost is unlikely to drop to the level of other inexpensive long-duration energy storage systems (>12 hours). To make long-duration energy storage applications more affordable, the U.S. Department of Energy should support research, development, and demonstration to advance electrochemical energy storage technologies that rely on abundant material substitution.
The cost, lifetime, and fabrication scale requirements of long-duration energy storage systems facilitate the exploration of novel electrochemical technologies, such as redox flow batteries and metal-air batteries, which use inexpensive materials and battery design applications that are more suitable for longer-duration energy storage applications. While several novel electrochemical technologies have shown promising development, knowledge gaps remain on key scientific, engineering, and manufacturing challenges, suggesting the high value of coordinated government support. Other countries, especially China, are actively pursuing innovations in these technologies.
6. Thermal energy storage
Thermal energy storage (TES) has properties suitable for long-term energy storage, including the ability to efficiently store heat in low-cost materials. This research report discusses several common thermal energy storage (TES) strategies that reflect varying levels of technology. One possible thermal energy storage (TES) approach focuses on replacing existing fossil-fueled boilers by reusing steam turbines in existing power plants and adding heat storage and steam generators, thereby reducing the conversion of thermal energy to electricity, which is the main cost component of thermal energy storage (TES) systems. This retrofit, which can now be done using economically viable technologies, could be attractive to factories and local communities as a way to use assets that would otherwise be obsolete as the power system decarbonizes .
7. Chemical energy storage
Hydrogen is widely regarded as a leading chemical energy storage medium because it can be produced directly from electricity in simple steps and used as a fuel for power generation or as a feedstock or heat source for other industrial processes. The research project therefore focuses on hydrogen in the chemical energy storage section. The role of hydrogen as a form of energy storage in the power sector may depend on the extent to which hydrogen is used in the overall economy and the cost of producing, transporting and storing it in the future, as well as the pace of innovation in hydrogen uses.
Hydrogen is currently produced, transported, and sold as a feedstock for many industrial processes. Today, major hydrogen production technologies rely on fossil fuel production and produce carbon emissions. The ability to use low-carbon electricity to produce low-carbon hydrogen from water electrolysis can support the decarbonization of end-use sectors such as industry and transportation, as well as the power sector. Figure 2 shows how hydrogen produced by electrolysis can be used as a low-carbon fuel for industry and power generation during periods of low renewable energy generation. Using electrolyzers as dispatchable loads on the power system can also reduce the cost of power system decarbonization by increasing the capacity utilization of renewable energy resources.
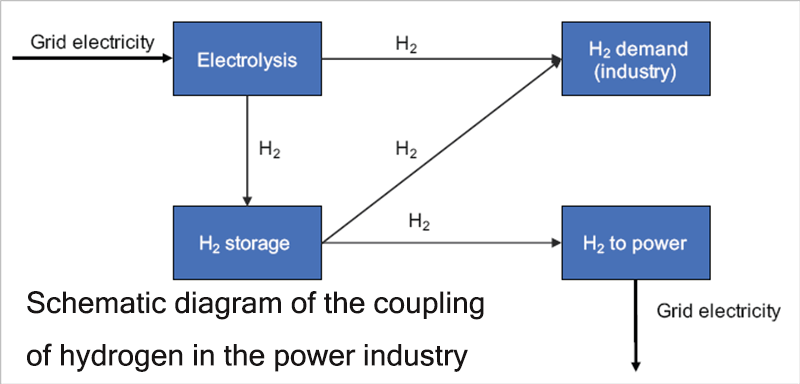
Figure 2 Schematic diagram of the coupling of hydrogen in the power industry
The research team is committed to supporting the U.S. Department of Energy's efforts to develop a national strategy to address hydrogen production, transportation, and storage. In particular, whether existing natural gas pipelines can deliver hydrogen unaffected, either under reduced pressure, or when hydrogen is mixed with natural gas or other compounds, remains an open question. Government-supported research is required by the U.S. Department of Energy and the U.S. Department of Transportation. An important step in this direction is recent legislation calling for at least four hydrogen production hubs in the United States.
8. Mechanical energy storage
Electrical energy can be converted into various forms of mechanical energy such as gravitational potential energy and kinetic energy. Electricity can also be used to compress gases such as air. Some of these forms of mechanical energy are suitable for large-scale and long-duration energy storage. As a category, mechanical energy storage includes a variety of technologies. However, a common feature of all these technologies is that their energy density is much lower than that of chemical or electrochemical energy storage technologies.
As a result, mechanical energy storage systems tend to have large footprints and high requirements on location and conditions, making them less suitable for use in small facilities. A pumped storage facility (PSH) stores energy in an upstream reservoir. Pumped storage hydro (PSH) is a widely deployed and mature energy storage technology, accounting for more than 90% of the grid-scale energy storage capacity currently in existence around the world and in the United States. However, since the 1990s, the deployment of pumped storage power generation (PSH) has slowed significantly in the United States and other countries.
Among other factors, this trend reflects the lower value of intraday energy arbitrage due to greater use of flexible natural gas for power generation. Additionally, pumped storage (PSH) projects have high initial investment costs and inflexible scale and siting requirements. Historically, these projects have also experienced lengthy construction cycles and significant cost overruns. While not strictly an electrical energy storage technology, existing conventional hydropower facilities could play a greater role in balancing supply and demand in a power system that relies heavily on renewable energy sources.
Where there is significant potential to play this role, power system planners should consider increasing the amount of water that reservoirs use to balance the power system. Advanced Compressed Air Energy Storage (CAES) systems store compressed air in underground caverns or above-ground storage tanks, and some Advanced Compressed Air Energy Storage (CAES) systems can also store the heat generated when the air is compressed. The technology has been widely discussed as a potential grid-scale energy storage system, but it faces significant hurdles to large-scale deployment.
Despite multiple uncertainties in cost estimates for advanced compressed air energy storage (CAES), the cost of the technology is generally higher than the cost of energy storage technologies available in the future. Co-locating energy storage systems with existing power plants that are being decommissioned can reduce storage costs through shared use of existing grid interconnection facilities and, in some cases, other power plant facilities. Using existing interconnection facilities will save time and cost.
Additionally, as mentioned above, existing fossil fuel turbines can be reused in thermal energy storage systems that use zero-emission heat or fuel to power existing turbines. The DOE should investigate the cost and system impacts of thermal energy storage technologies, as well as other promising options for reusing existing assets, and the social acceptance of such reuse strategies by neighboring communities, and should sponsor demonstration projects where appropriate.
9. Efficient renewable energy power systems with energy storage
This section explores the potential role of energy storage systems in the context of developing economies in both developed and emerging markets. The results for three different regions in the United States and India illustrate the deployment of energy storage in these two countries, respectively.
① Modeling results for developed countries: three regions in the United States
The research team's modeling of the U.S. power sector focused on three regions: the Northeast (New York and New England), the Southeast, and Texas with the latest deployments of energy storage in 2050. These regions differ significantly in electricity demand profiles, wind and solar generation resources, and availability of hydropower and existing nuclear power resources. These differences affect the lowest-cost generation mix without carbon caps and the cost of achieving varying degrees of decarbonization.
Figure 3 shows simulated projections of annual electricity generation, deliverable energy capacity, and power system electricity costs for each region in 2050 under two policy scenarios: no carbon cap and emissions capped at 5 g carbon emissions/kWh. If electricity demand in 2050 is the same as in 2018, reducing the average carbon intensity of the U.S. power sector to 5 grams of carbon emissions per kWh would reduce carbon emissions in 2050 by 99.2% compared to 2005 levels.

Figure 3 Annual power generation relative to electricity demand
On the other hand, if the demand for electricity consumption growth in 2050 is greater than that in 2018, as predicted in the electricity demand scenario used in the study to simulate the impact of energy storage systems. Average carbon emissions across U.S. industries compared to 2005, with electricity sector emissions down 98.7 percent. Figure 3 illustrates the results from scenarios assuming only lithium-ion batteries and pumped hydro storage are available, and the research team’s modeling of the U.S. region evaluated a wide range of other energy storage technologies.
The ability of energy storage to replace or complement all other elements of the power system (including generation, transmission, and demand response), coupled with the uncertain impact of climate change on electricity demand and supply, means more sophisticated analytical tools are required. Future power systems need to be planned, operated, and regulated, as well as to ensure power system reliability and efficiency.
Important areas of focus include power system stability and dispatch (including enabling participation and compensation of distributed storage and generation assets in system dispatch and wholesale markets), resource adequacy, and retail rate design. The development of new analytical tools must be accompanied by additional support for complementary staffing and upskilling programmes for regulators. This work should be undertaken by the DOE in partnership with Independent System Operators and Regional Transmission Organizations (ISO/RTOs).
In a deeply decarbonized power system, the distribution of hourly wholesale prices, or the marginal value of energy, will change, with much more time at zero or very low prices for electricity than in the current wholesale market, with high prices Much more time. This is because, compared with the current power system that mainly relies on thermal power generation facilities, a power system with energy storage systems based on renewable energy will have relatively high fixed investment costs and relatively low marginal operating costs.
Figure 4 compares the distribution of hourly wholesale electricity prices in 2018 and 2019 for the Electric Reliability Commission of Texas (ERCOT) power system, which covers nearly all of Texas, and 2050 year's scenario. The bars in the graph represent the price distribution for the unrestricted and carbon-restricted Texas modeled cases. Increased reliance on renewable energy generation facilities at zero marginal cost. This effect increases as carbon restrictions become stricter (i.e. allowable emissions are progressively lower). During the period of highest price, as shown by the top of the bar and the breakdown of the graph, the simulated price was significantly higher than the current Electric Reliability Commission of Texas (ERCOT) market price.
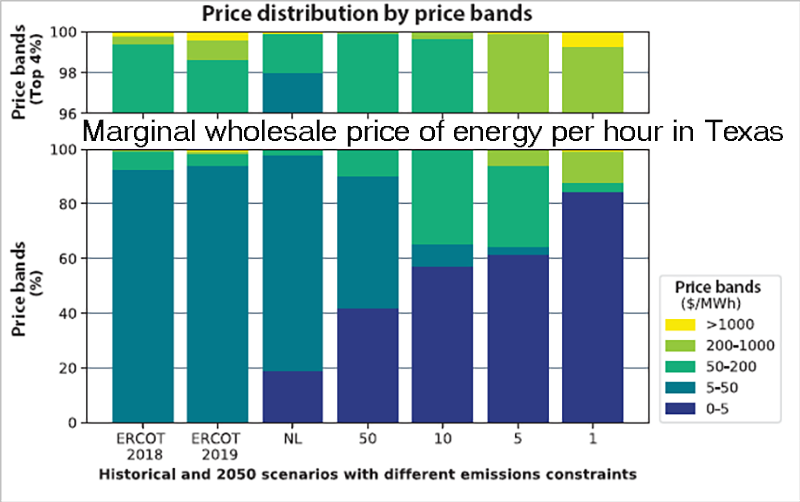
Figure 4 Marginal wholesale price of energy per hour in Texas
The combination of relatively high capital costs and longer periods of time at very low prices will create financing challenges for renewable energy generation and storage. Especially since regulators will likely continue to cap (as they currently do) extremely high prices that might otherwise support cost recovery. The future model of wholesale electricity pricing and the goal of decarbonizing other industries through the electrification of decarbonized electricity also reinforce the benefits of adopting retail pricing and retail load management options.
Allows for increased deployment of renewable energy in better locations and improves renewable energy integration by balancing resource intermittency between connected regions and reducing the impact of geographic disparities in renewable energy supply and demand, which is essential for cost-effective decarbonization also important. Currently, the potential for cost-effective transmission projects to bring electricity from regions rich in renewables to major load centers will face extended delays or cancellations, suggesting that legal and regulatory changes are needed to reduce barriers to transmission expansion. Insufficient transmission capacity could lead to a greater role for energy storage in the future and higher costs for the power system in the future.
② Modeling results for emerging market and developing economies: India
Research shows that emerging markets and developing economies that rely on coal-fired power generation do not have access to abundant low-cost natural gas infrastructure, such as India representing a very large and important future market for energy storage technology power system applications. Modelling in this study shows that energy storage systems will be deployed primarily at the transmission level, with important additional applications in urban distribution networks.
Overall economic growth (especially the rapid adoption of air conditioners in India) will be the main driver of energy storage deployment. Assuming a continued decline in technology costs, it is found that from a cost perspective, India's renewable energy generation facilities have an advantage in competing with coal-fired power plants in the medium to long term, but existing coal-fired power plants still lack carbon pricing, as shown in Figure 5 left side shown.
The right side of Figure 5 shows the modelling results for a scenario assuming that low-cost energy storage systems and renewable power generation technologies are available in India. These results show a significant reduction in both system cost and modelled CO2 emissions from the Indian power system compared to baseline projections (see graph on the left). Electricity system costs and CO2 emissions are reduced regardless of carbon caps or taxes. This result highlights the global environmental benefits of reducing the cost of energy storage.
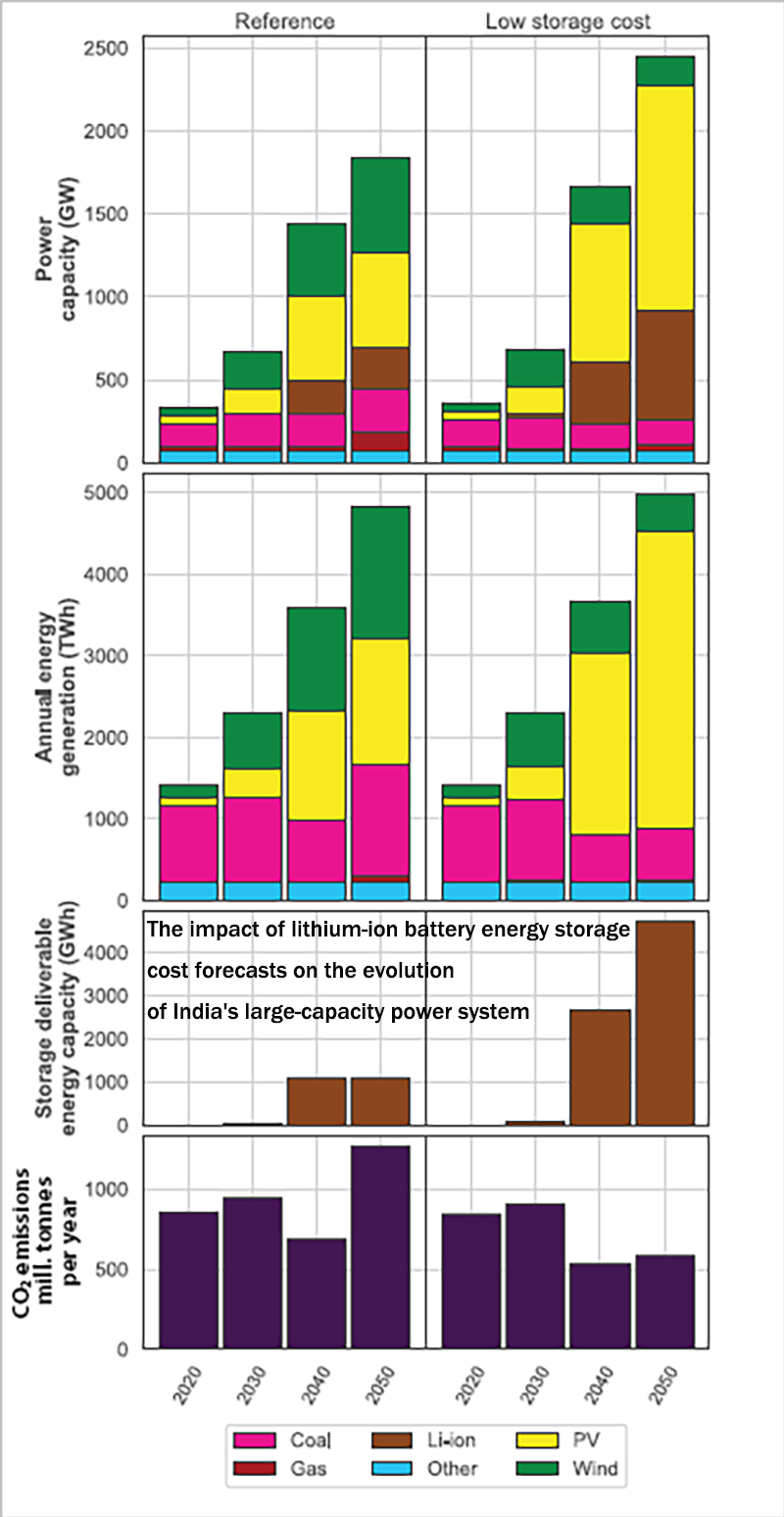
Figure 5 The impact of lithium-ion battery energy storage cost forecasts on the evolution of India's large-capacity power system
10. Other research topics
In addition to the topics discussed in this study, there are several energy storage systems-related topics that deserve attention. These include: (1) Manufacturing and supply chain trends, implications for the availability and cost of energy storage technologies and U.S. competitiveness. (2) The relationship between the stability of economic and regulatory policy frameworks for economy-wide decarbonization and the time required to achieve a net-zero carbon power sector. (3) Establish expectations for end-of-life battery recovery and reuse. (4) Environmental identification, health and safety aspects of specific energy storage systems including home energy storage. (5) The practical availability range of load flexibility and demand response to reduce energy storage demand and associated costs.